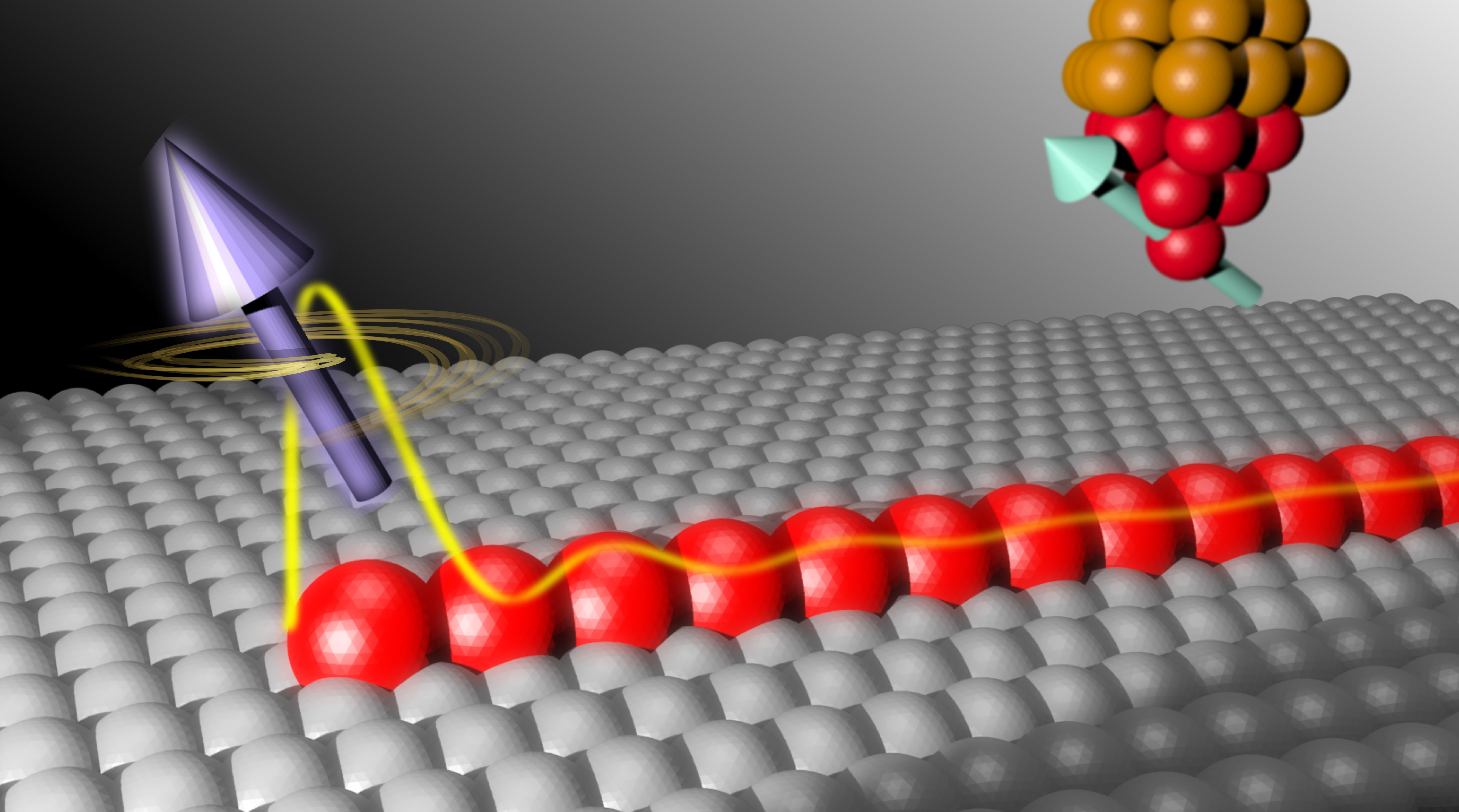
By Catherine Zandonella, Office of the Dean for Research
Researchers at Princeton University have detected a unique quantum property of an elusive particle notable for behaving simultaneously like matter and antimatter. The particle, known as the Majorana fermion, is prized by researchers for its potential to open the doors to new quantum computing possibilities.
In the study published this week in the journal Science, the research team described how they enhanced an existing imaging technique, called scanning tunneling microscopy, to capture signals from the Majorana particle at both ends of an atomically thin iron wire stretched on the surface of a crystal of lead. Their method involved detecting a distinctive quantum property known as spin, which has been proposed for transmitting quantum information in circuits that contain the Majorana particle.
“The spin property of Majoranas distinguishes them from other types of quasi-particles that emerge in materials,” said Ali Yazdani, Princeton’s Class of 1909 Professor of Physics. “The experimental detection of this property provides a unique signature of this exotic particle.”
The finding builds on the team’s 2014 discovery, also published in Science, of the Majorana fermion in a single atom-wide chain of iron atoms atop a lead substrate. In that study, the scanning tunneling microscope was used to visualize Majoranas for the first time, but provided no other measurements of their properties.
“Our aim has been to probe some of the specific quantum properties of Majoranas. Such experiments provide not only further confirmation of their existence in our chains, but open up possible ways of using them.” Yazdani said.
First theorized in the late 1930s by the Italian physicist Ettore Majorana, the particle is fascinating because it acts as its own antiparticle. In the last few years, scientists have realized that they can engineer one-dimensional wires, such as the chains of atoms on the superconducting surface in the current study, to make Majorana fermions emerge in solids. In these wires, Majoranas occur as pairs at either end of the chains, provided the chains are long enough for the Majoranas to stay far enough apart that they do not annihilate each other. In a quantum computing system, information could be simultaneously stored at both ends of the wire, providing a robustness against outside disruptions to the inherently fragile quantum states.
Previous experimental efforts to detect Majoranas have used the fact that it is both a particle and an antiparticle. The telltale signature is called a zero-bias peak in a quantum tunneling measurement. But studies have shown that such signals could also occur due to a pair of ordinary quasiparticles that can emerge in superconductors. Professor of Physics Andrei Bernevig and his team, who with Yazdani’s group proposed the atomic chain platform, developed the theory that showed that spin-polarized measurements made using a scanning tunneling microscope can distinguish between the presence of a pair of ordinary quasi-particles and a Majorana.
Typically, scanning tunneling microscopy (STM) involves dragging a fine-tipped electrode over a structure, in this case the chain of iron atoms, and detecting its electronic properties, from which an image can be constructed. To perform spin-sensitive measurements, the researchers create electrodes that are magnetized in different orientations. These “spin-polarized” STM measurements revealed signatures that agree with the theoretical calculations by Bernevig and his team.
“It turns out that, unlike in the case of a conventional quasi-particle, the spin of the Majorana cannot be screened out by the background. In this sense it is a litmus test for the presence of the Majorana state,” Bernevig said.
The quantum spin property of Majorana may also make them more useful for applications in quantum information. For example, wires with Majoranas at either end can be used to transfer information between far away quantum bits that rely on the spin of electrons. Entanglement of the spins of electrons and Majoranas may be the next step in harnessing their properties for quantum information transfer.
The STM studies were conducted by three co-first authors in the Yazdani group: scientist Sangjun Jeon, graduate student Yonglong Xie, and former postdoctoral research associate Jian Li (now a professor at Westlake University in Hangzhou, China). The research also included contributions from postdoctoral research associate Zhijun Wang in Bernevig’s group.
This work has been supported by the Gordon and Betty Moore Foundation as part of the EPiQS initiative (grant GBMF4530), U.S. Office of Naval Research (grants ONR-N00014-14-1-0330, ONR-N00014-11-1-0635, and ONR- N00014-13-1-0661) , the National Science Foundation through the NSF-MRSEC program (grants DMR-142054 and DMR-1608848) and an EAGER Award (grant NOA -AWD1004957), the U.S. Army Research Office MURI program (grant W911NF-12-1-046), the U.S. Department of Energy Office of Basic Energy Sciences, the Simons Foundation, the David and Lucile Packard Foundation, and the Eric and Wendy Schmidt Transformative Technology Fund at Princeton.
The study, “Distinguishing a Majorana zero mode using spin resolved measurements,” was published in the journal Science on Thursday, October 12, 2017.
You must be logged in to post a comment.