By Catherine Zandonella, Office of the Dean for Research
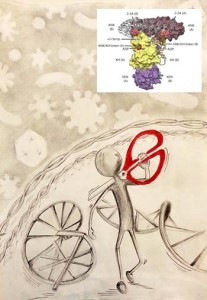
An essential weapon in the body’s fight against infection has come into sharper view. Researchers at Princeton University have discovered the 3D structure of an enzyme that cuts to ribbons the genetic material of viruses and helps defend against bacteria.
The discovery of the structure of this enzyme, a first-responder in the body’s “innate immune system,” could enable new strategies for fighting infectious agents and possibly prostate cancer and obesity. The work was published Feb. 27 in the journal Science.
Until now, the research community has lacked a structural model of the human form of this enzyme, known as RNase L, said Alexei Korennykh, an assistant professor of molecular biology and leader of the team that made the discovery.
“Now that we have the human RNase L structure, we can begin to understand the effects of carcinogenic mutations in the RNase L gene. For example, families with hereditary prostate cancers often carry genetic mutations in the region, or locus, encoding RNase L,” Korennykh said. The connection is so strong that the RNase L locus also goes by the name “hereditary prostate cancer 1.” The newly found structure reveals the positions of these mutations and explains why some of these mutations could be detrimental, perhaps leading to cancer, Korennykh said. RNase L is also essential for insulin function and has been implicated in obesity.
The Princeton team’s work has also led to new insights on the enzyme’s function.
The enzyme is an important player in the innate immune system, a rapid and broad response to invaders that includes the production of a molecule called interferon. Interferon relays distress signals from infected cells to neighboring healthy cells, thereby activating RNase L to turn on its ability to slice through RNA, a type of genetic material that is similar to DNA. The result is new cells armed for destruction of the foreign RNA.
The 3D structure uncovered by Korennykh and his team consists of two nearly identical subunits called protomers. The researchers found that one protomer finds and attaches to the RNA, while the other protomer snips it.
The initial protomer latches onto one of the four “letters” that make up the RNA code, in particular, the “U,” which stands for a component of RNA called uridine. The other protomer “counts” RNA letters starting from the U, skips exactly one letter, then cuts the RNA.
Although the enzyme can slice any RNA, even that of the body’s own cells, it only does so when activated by interferon.
“We were surprised to find that the two protomers were identical but have different roles, one binding and one slicing,” Korennykh said. “Enzymes usually have distinct sites that bind the substrate and catalyze reactions. In the case of RNase L, it appears that the same exact protein surface can do both binding and catalysis. One RNase L subunit randomly adopts a binding role, whereas the other identical subunit has no other choice but to do catalysis.”
To discover the enzyme’s structure, the researchers first created a crystal of the RNase L enzyme. The main challenge was finding the right combination of chemical treatments that would force the enzyme to crystallize without destroying it.
After much trial and error and with the help of an automated system, postdoctoral research associate Jesse Donovan and graduate student Yuchen Han succeeded in making the crystals.
Next, the crystals were bombarded with powerful X-rays, which diffract when they hit the atoms in the crystal and form patterns indicative of the crystal’s structure. The diffraction patterns revealed how the atoms of RNase L are arranged in 3D space.
At the same time Sneha Rath, a graduate student in Korennykh’s laboratory, worked on understanding the RNA cleavage mechanism of RNase L using synthetic RNA fragments. Rath’s results matched the structural findings of Han and Donovan, and the two pieces of data ultimately revealed how RNase L cleaves its RNA targets.
Han, Donovan and Rath contributed equally to the paper and are listed as co-first authors.
Finally, senior research specialist Gena Whitney and graduate student Alisha Chitrakar conducted additional studies of RNase L in human cells, confirming the 3D structure.
Now that the human structure has been solved, researchers can explore ways to either enhance or dampen RNase L activity for medical and therapeutic uses, Korennykh said.
“This work illustrates the wonderful usefulness of doing both crystallography and careful kinetic and enzymatic studies at the same time,” said Peter Walter, professor of biochemistry and biophysics at the University of California-San Francisco School of Medicine. “Crystallography gives a static picture which becomes vastly enhanced by studies of the kinetics.”
Support for the work was provided by Princeton University.
Han, Yuchen, Jesse Donovan, Sneha Rath, Gena Whitney, Alisha Chitrakar, and Alexei Korennykh. Structure of Human RNase L Reveals the Basis for Regulated RNA Decay in the IFN Response Science 1249845. Published online 27 February 2014 [DOI:10.1126/science.1249845]
You must be logged in to post a comment.