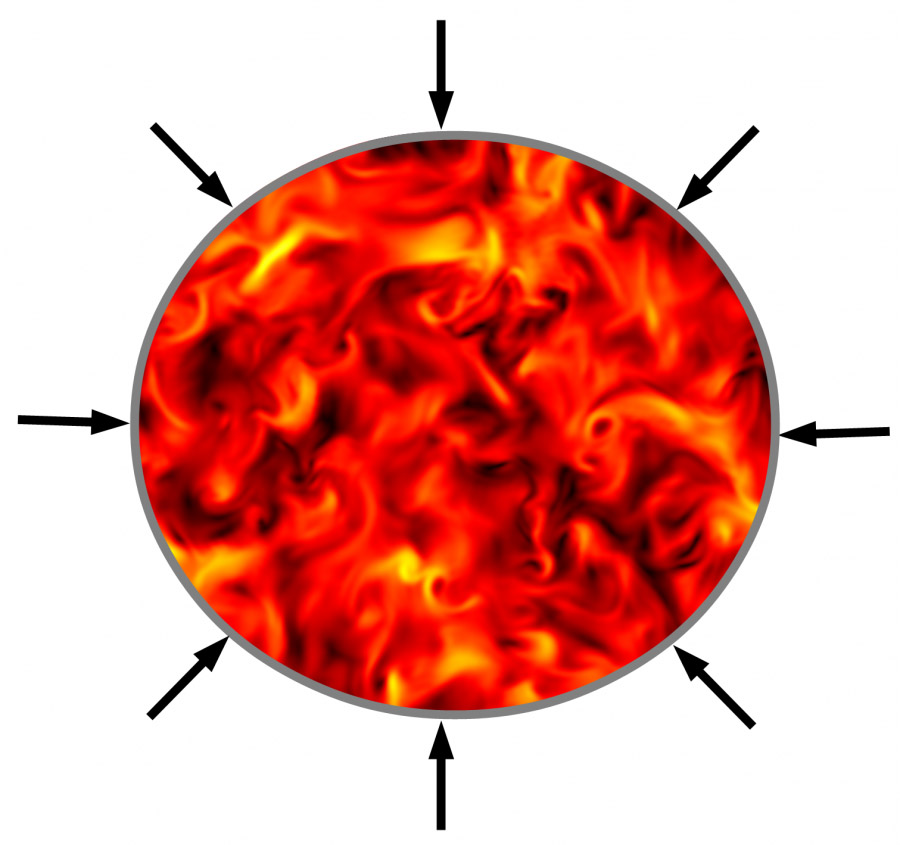
By John Greenwald, Princeton Plasma Physics Laboratory
Physicists have long regarded plasma turbulence as unruly behavior that can limit the performance of fusion experiments. But new findings by researchers associated with the U.S. Department of Energy’s (DOE) Princeton Plasma Physics Laboratory (PPPL) and the Department of Astrophysical Sciences at Princeton University indicate that turbulent swirls of plasma could benefit one of the two major branches of such research. The editors of Physical Review Letters highlighted these findings — a distinction given to one of every six papers per issue — when they published the results last week on March 11.
Lead author Seth Davidovits, a Princeton University graduate student, and Professor of Astrophysical Sciences Nathaniel Fisch, his thesis adviser and Associate Director for Academic Affairs at PPPL, produced the findings. They modeled the compression of fluid turbulence, showing effects that suggested a surprising positive impact of turbulence on inertial confinement fusion (ICF) experiments.
Stimulating this work were experiments conducted by Professor Yitzhak Maron at the Weizmann Institute of Science in Israel. Those experiments, on a Z-pinch inertial confinement machine, showed turbulence that contained a surprising amount of energy, which caught Fisch’s attention during a recent sabbatical at Weizmann.
In a Z-pinch and other inertial confinement (ICF) machines, plasma is compressed to create fusion energy. The method contrasts with the research done at PPPL and other laboratories, which controls plasma with magnetic fields and heats it to fusion temperatures in doughnut-shaped devices called tokamaks. The largest Z-pinch device in the United States is at the DOE’s Sandia National Laboratory. Other inertial confinement approaches are pursued at, among other places, the DOE’s Lawrence Livermore National Laboratory.
Present ICF approaches use compression to steadily heat the plasma. Methods range from squeezing plasma with magnetic fields at Sandia to firing lasers at capsules filled with plasma at Livermore’s National Ignition Facility. The presence of turbulence in the plasma is widely thought to increase the difficulty of achieving fusion.
But there could be advantages to turbulence if handled properly, the authors point out, since energy contained in turbulence does not radiate away. This compares with hotter plasmas in which heat radiates away quickly, making fusion harder to achieve. By storing the energy of the compression in turbulence rather than temperature, the authors suppress the energy lost to radiation during the compression.
The turbulent energy also does not immediately lead to fusion, which requires high temperature. This means a mechanism is needed to change the turbulence into the temperature required for fusion once the plasma has been compressed.
Davidovits used a software code called Dedalus to show that turbulent energy is increased during the compression, but then suddenly transformed into heat. As external forces in his simulation compress the turbulence to increase the energy stored within it, they also gradually raise the temperature and viscosity of the plasma. The viscosity, which describes how “thick” or resistant to flow a fluid is, acts to slow the turbulence and convert its energy to temperature. The viscosity started small so that the turbulence was initially unhindered. The rapid compression then kept the viscosity growing until it suddenly catalyzed the transfer of energy from the turbulence to the temperature.
In an experiment, this process would create the conditions for nuclear fusion in a plasma composed of the hydrogen isotopes deuterium and tritium. “This suggests a fundamentally different design for compression-based fusion experiments,” Davidovits said, “and a new paradigm for the inertial technique of producing fusion energy.”
He warns, however, that the simulation includes caveats that could diminish the findings. For example, the model doesn’t consider any possible interaction between the plasma and the containing capsule, and highly energetic turbulence might mix parts of the capsule into the plasma and contaminate the fusion fuel.
Nonetheless, the authors call the rapid transfer of turbulent energy into temperature during ICF experiments a “tantalizing” prospect that could benefit such research. And they note that their findings could lead to new understanding of the evolution of the relationship between the pressure, volume and temperature of a gas that is substantially turbulent. Determining this will be quite challenging, they say, “but the understanding will be important not only for the new fusion approach, but also for many situations involving the behavior of low viscosity compressible fluids and gases.”
This research was initiated through a grant by the Defense Threat Reduction Agency, a unit of the U.S. Department of Defense, and has been supported also by the DOE’s National Nuclear Security Administration through a consortium with Cornell University. Recently, the National Science Foundation and the Israel Binational Science Foundation combined funding opportunities to ensure further experiments at Weizmann on this topic and continued collaboration with the Princeton researchers.
PPPL, on Princeton University’s Forrestal Campus in Plainsboro, N.J., is devoted to creating new knowledge about the physics of plasmas — ultra-hot, charged gases — and to developing practical solutions for the creation of fusion energy. Results of PPPL research have ranged from a portable nuclear materials detector for anti-terrorist use to universally employed computer codes for analyzing and predicting the outcome of fusion experiments. The Laboratory is managed by the University for the U.S. Department of Energy’s Office of Science, which is the largest single supporter of basic research in the physical sciences in the United States, and is working to address some of the most pressing challenges of our time. For more information, please visit science.energy.gov.
Read the abstract or paper here.
S. Davidovits, N. Fisch, Sudden Viscous Dissipation of Compressing Turbulence. Phys. Rev. Lett. 116, 105004 – Published 11 March 2016.
This work was supported by DOE through Contracts No. DE-AC02-09CH1-1466 and NNSA 67350-9960 (Prime No. DOE DE-NA0001836), by DTRA HDTRA1-11-1-0037, and by NSF Contract No. PHY-1506122.
You must be logged in to post a comment.